The electron microscope is a type of microscope that uses a beam of electron to create an image of the specimen. It is capable of much higher magnifications and has a greater resolving power than a light microscope, allowing it to see much smaller objects in finer details. They are large, expensive pieces of equipment, generally standing alone in a small, specially designed room and requiring trained personnel to operate them.
Applications
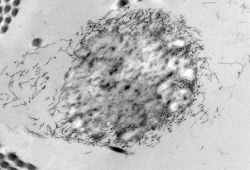
Imaging
Award winning, high resolution imaging tools help you to understand ultrastructure of biological and inorganic specimens.
Imaging - Read More
OVERVIEW
There are a number of options and technologies available for digital imaging in transmission electron microscopy (TEM) applications today. Traditionally, high energy electrons could not be directly exposed to a sensor without excessively damaging the detector. As a consequence, conventional TEM cameras first expose the incoming electron beam to a scintillating film that converts the electrons into light (photons). These photons are then transferred to the sensor, either through a series of optical lenses or a coupled fiber optic plate. Finally, the light is collected by a sensor where the image is created pixel-by-pixel based on the amount of light detected at each position in the sensor.
CONVENTIONAL TEM IMAGE DETECTION ARCHITECTURE
There are four basic steps in TEM imaging to address incoming electrons:
- Convert electrons to signal
- Transfer signal
- Detect signal with sensor
- Electronically transfer signal and read-out to form image
WHAT IS DIFFERENT WITH DIRECT DETECTION?
There are only two steps in TEM imaging with direct detection:
- Convert electrons to signal – not applicable
- Transfer signal – not applicable
- Detect signal with sensor
- Electronically transfer signal and read-out to form image
One key difference between conventional and direct detection is a custom CMOS sensor that utilizes the only radiation hard architecture that can tolerate direct exposure to high energy particles. To add, extremely high speed electronics for data transfer and processing enable low-dose counting and super-resolution capabilities. Combined, this allows frame rates (4k x 4k) of 400 frames per second (fps) to be processed in real-time to achieve optimal results.
STEP 1) CONVERT ELECTRONS TO SIGNAL
Gatan uses proprietary phosphor scintillators to optimize signal conversion that enhances detector sensitivity (SENS) and resolution. When you select a scintillator, it is appropriate to know the performance trade-offs between SENS and resolution.
- Sensitivity (signal): Ideal for dose-sensitive use cases where you need to generate more photons per incoming electron (e.g., cryo-tomography, beam sensitive materials)
- Resolution (spatial detail): Favorable for applications where you require more information to resolve details, but you can increase the dose (signal) without harming the sample (e.g., semiconductors and other less-sensitive materials)
STEP 2) TRANSFER SIGNAL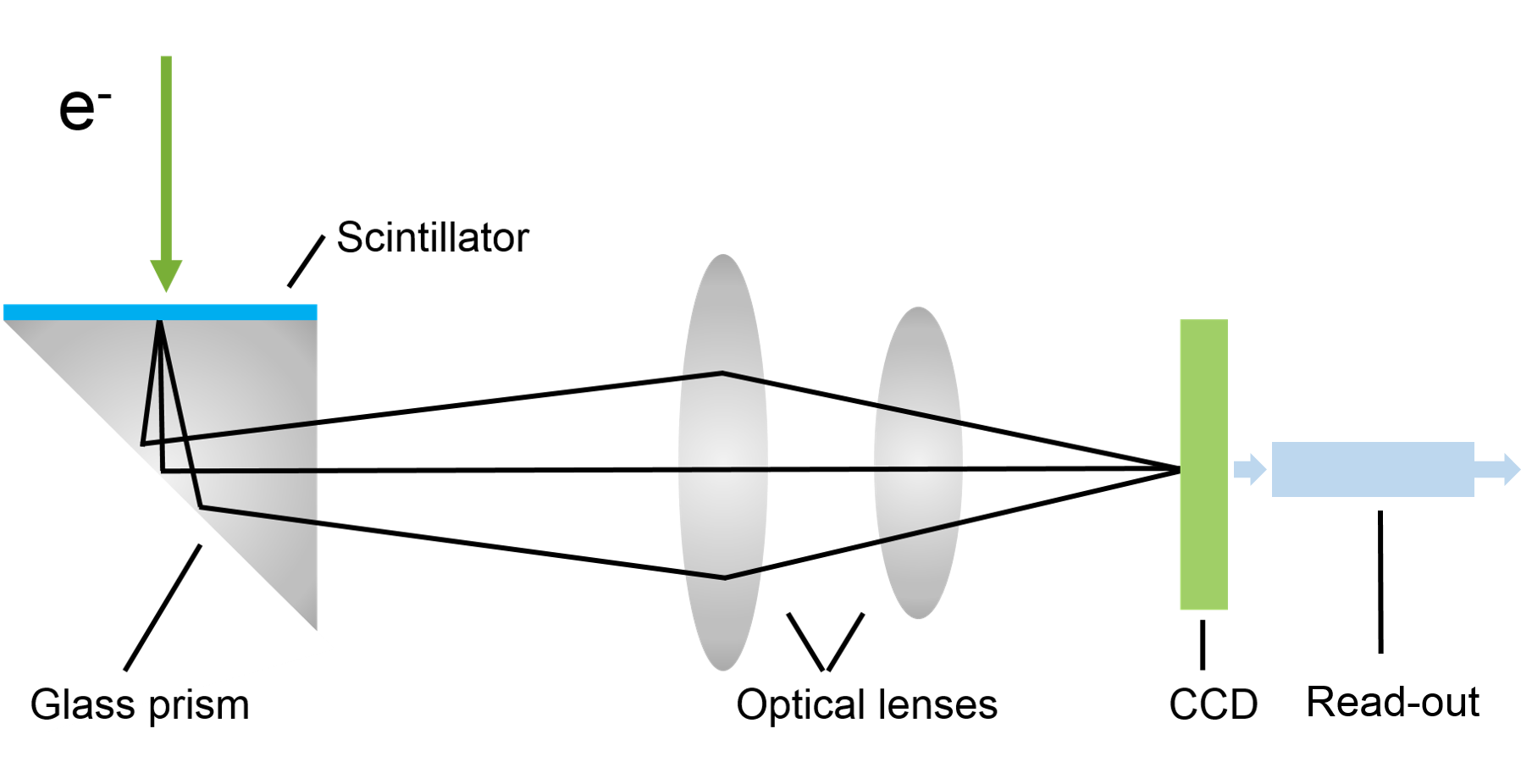
Various coupling (lens- and fiber-coupled) mechanisms are available to optimize signal transfer and meet cost or performance targets for a given detector.
Lens-coupled: Lens optics transmit light to the sensor where it is converted into sensor electrons (signal)
- Pros: (Gatan) Uses real transmission scintillator; can be less expensive than higher performance fibers
- Cons: Light (information) loss with lensing, angular dependencies (<10% efficient); vignetting (light fall-off); image distortion for higher magnification
Fiber-coupled: Scintillator creates photons that subsequently create sensor electrons; fiber directly transmits light to sensor with high efficiency
- Pros: Most efficient transmission of light information to the sensor (1:1 coupling of scintillator:sensor (>50% efficient) with no image distortion); can trade-off SENS verses resolution (fiber configuration detail)
- Cons: Fiber optics are slightly higher cost; requires process optimization including cladding, sintering, bonding of fibers (Gatan proprietary)
Renal sample at 3000x magnification. Fiber-coupled (left) vs. lens-coupled (right).
STEP 3) DETECT SIGNAL WITH SENSOR
Sensor type (CCD vs. CMOS) offers significant trade-offs for TEM camera performance as there are fundamental differences in architecture.
- Charge-coupled device (CCD): Charge transfers between neighboring cells, and read-out (e.g., noise) is seen at final stage; binning minimizes the impact of read-out noise
- Complementary metal–oxide–semiconductor (CMOS): Charge immediately converts to voltage (read-out with digital output); supports high frame rates, low overall electronics noise
Both technologies possess inherent advantages, so the question arises about what unique performance characteristics arise from each choice. CCDs can have 100% fill factor that captures all incoming light, whereas part of the CMOS sensor is occupied by transistors and metal wiring associated with each pixel. Historically, CCDs provided higher quality images with low noise, at affordable prices. Recent design advancements and processing techniques now advance CMOS sensor performance so it is a viable choice for some applications. Note that CCDs still maintain an advantage for binning in terms of signal-to-noise. However, CMOS chips can scale the number of read-out ports and achieve very high frame rates.
Image courtesy of http://meroli.web.cern.ch/meroli/lecture_cmos_vs_ccd_pixel_sensor.html
STEP 4) TRANSFER SIGNAL AND READ-OUT IMAGE
When a charge converts to voltage, you typically generate noise
- CCD: Transfer data out of serial register
- CMOS: Converts to voltage per pixel
It is very important to optimize read-out noise (higher voltages) and speed (multi-port and fast read times) for CCDs.
- Optimize controller for low read-noise; leverage multi-port read-outs for faster speed
- Interline CCDs with binning have the fastest readouts (fps) – up to 30 fps due to 100% duty cycle
CMOS typically is seen as a fast sensor because you can run in rolling shutter mode verses the slow global shutter mode.
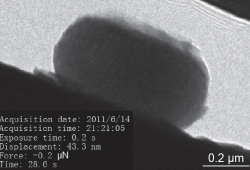
In-Situ
Real-time observation of growth processes, chemical reactions and oxidation, irradiation effects, mechanical, magnetic, and ferroelectric properties.
In-Situ - Read More
OVERVIEW
What is in-situ microscopy?
In-situ transmission electron microscopy combines the image formation capabilities of the transmission electron microscope (TEM) with application of some external stimulus to measure results real-time. Currently, a wide variety of systems and holders are available to apply different stimuli to evaluate kinetic reactions such as catalysis, phase transformations, other chemical reactions, electrical fields, mechanical strain or nano-indentation deformation, heating and cooling, or the effects of the electron beam irradiation damage in samples.
Historically, movies of various reactions and system kinetics could be recorded onto video tape. More recently screen capture programs allow low-resolution, qualitative capture for you to visualize what is happening at video frame rates, e.g., 30 frames per second (fps). This adds significant value to investigators who want to physically see and understand reactions in real-time.
With the advent of faster data transfer and processing capabilities, it is now possible to record and manage large datasets directly from the sensor output. You can store each pixel in each frame directly to disk, and treat them as an individual image. Alternatively you can apply various algorithms or scripts (e.g., summing, drift correction, binning, etc.) post-capture to quantify the stimulus applied to the system. This greatly enhances the flexibility of data management and gives you previously unseen resolution in time and space, with sub-ms time resolution to resolve reactions that previously were unknown.
PROTOCHIPS TECHNOLOGY
Their Technology
Their Technology
For over a decade, Protochips pioneered electron microscopy solutions to accelerate your in situ research. Protochips innovation is accomplished by continuously focusing on your needs and constantly improving your experience through our products. Learn more about the innovations at the core of your product and how they've improved the in situ microscopy experience below.
Isolated Electrical Contacts - Using precisely located electrical leads, these E-chip designs deliver electrical or thermal biasing capabilities regardless of the experimental set-up.
Precise Electrical Delivery - Imbedded electrical leads, self-aligning parts, and secure electrical connection throughout the tilt range minimizes electical noise and maximizes the accuracy of electrical measurements.
Superior Thermal Control - Utilizing a chemically inert SiC heating membrane that doesn’t degrade at high temperatures, this technology enables accurate and uniform sample heating across the viewing area.
EDS-Optimized E-Chips - Minimizing the amount of material between the sample and detector, this process maximizes EDS Counts Per Second (CPS).
Closed-Cell Holder Design - The closed-cell holder design was one of the innovations that brought in situ techniques to numerous areas of research, enabling high resolution sample imaging in liquid and gaseous environments which was previously impossible. Using two self-aligning E-chips to safely encapsulate samples, this holder design transforms any TEM or STEM into a nanoscale in situ laboratory.
EDS-Optimised Holder - This ““butterfly lid”” design improves EDS counts per second (CPS) by utilizing a streamlined profile that allows for direct-line-of-sight to detectors, enabling faster EDS acquisition at any sample tilt.
Faster Tip Assembly - This single O-ring design aligns and perfectly seals E-chips to form a closed-cell that can be assembled in less than 5 minutes.
Safe Electrical Control - By isolating electrical contacts from the sealed liquid or gaseous environment, these chip designs enable quantitative analysis within the TEM.
Reliable Electrical Contacts - This design allows electrical connections within the holder to automatically align with imbedded electrical contacts within E-Chips, enabling easier sample loading and more accurate experiments.
Flat Carbon Support - This technique enables consistently flatter holey carbon films to be deposited onto standard sample support grids.
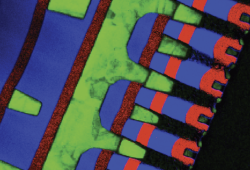
EELS
Electron energy loss spectroscopy (EELS) for chemical and compositional analysis.
EELS - Read More
OVERVIEW
TEM specimen beam interactions.Electron energy loss spectroscopy (EELS) is a family of techniques that measure the change in kinetic energy of electrons after they interact with a specimen. This technique is used to determine the atomic structure and chemical properties of a specimen, including: the type and quantity of atoms present, chemical state of atoms and the collective interactions of atoms with their neighbors. Some of these techniques include: spectroscopy, energy-filtered transmission electron microscopy (EFTEM), and DualEELS™.
Atom-scale view of electron energy loss.As electrons pass through a specimen, they interact with atoms of the solid. Many of the electrons pass through the thin sample without losing energy. A fraction will undergo inelastic scattering and lose energy as they interact with the specimen. This leaves the sample in an excited state. The material can de-excite by giving up energy typically in the form of visible photons, x-rays or Auger electrons.
As the incident electron interacts with the sample, it changes both its energy and momentum. You can detect this scattered incident electron in the spectrometer as it gives rise to the electron energy loss signal. The sample electron (or collective excitation) carries away this additional energy and momentum.
Core-loss excitations occur when tightly bound core electrons are promoted to a higher energy state by the incident electron. The core electron can only be promoted to an energy that is an empty state in the material. These empty sates can be bound states in the material above the Fermi level (so called anti-bonding orbitals in the molecular orbital picture). The states can also be free electron states above the vacuum level. It is the sudden turn-on of the scattering at the Fermi energy and the probing of empty states which makes the EELS signal sensitive to both the atom type and its electronic state.Correlation between EELS and specimen features.
You can visualize the initial spectral features in the core-loss excitations when you align the Fermi level with the zero-loss peak (ZLP) of the spectrum. The edges can now be seen as the point where the electrons lose enough energy to promote the core level atomic electrons to the Fermi level. This analogy fails to reproduce the scattering above the Fermi level, but is helpful to visualize the core level edge sudden increase in intensity.An EELS spectrum.
A typical energy loss spectrum includes several regions. The first peak, the most intense for a very thin specimen, occurs at 0 eV loss (equal to the primary beam energy) and is therefore called the zero-loss peak. It represents electrons that did not undergo inelastic scattering, but may have been scattered elastically or with an energy loss too small to measure. The width of the zero-loss peak mainly reflects the energy distribution of the electron source. It is typically 0.2 – 2.0 eV but may be as narrow as 10 meV or lower in a monochromated electron source.
For more information on the EELS family of techniques, please visit EELS.info, an educational site.
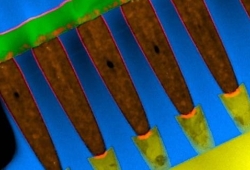
EFTEM
Energy-filtered transmission electron microscopy (EFTEM) is a family of imaging techniques to enhance, map and quantify elements and chemicals in an image.
EFTEM - Read More
OVERVIEW
Energy-filtered transmission electron microscopy (EFTEM) is a family of imaging techniques that utilize properties of the energy loss spectrum to increase contrast, remove the effects of chromatic aberration and create unique contrast effects in the image. Key applications include:
- Contrast enhancement – Improves contrast in images and diffraction patterns when it removes inelastically scattered electrons that produce background fog
- Including zero-loss filtering, most probable loss imaging, contrast tuning, and pre-carbon imaging
- Mapping – Creates elemental/chemical maps at nanometer resolution by forming images with inelastically scattered electrons
- Including 2- and 3-window elemental mapping/jump-ratio imaging and chemical mapping – provides fine structure imaging
- Analytical – Records and quantifies electron energy loss spectra (and maps) to provide chemical analysis of TEM samples
The principle behind EFTEM is based on the illumination of a very thin specimen with a beam of high energy electrons. When the majority electrons pass unhindered through the specimen, some will interact with the specimen and result in elastic or inelastic scattering. Inelastic scattering results in both a loss of energy and a change in momentum, which in the case of inner shell ionization is characteristic of the element in the sample.
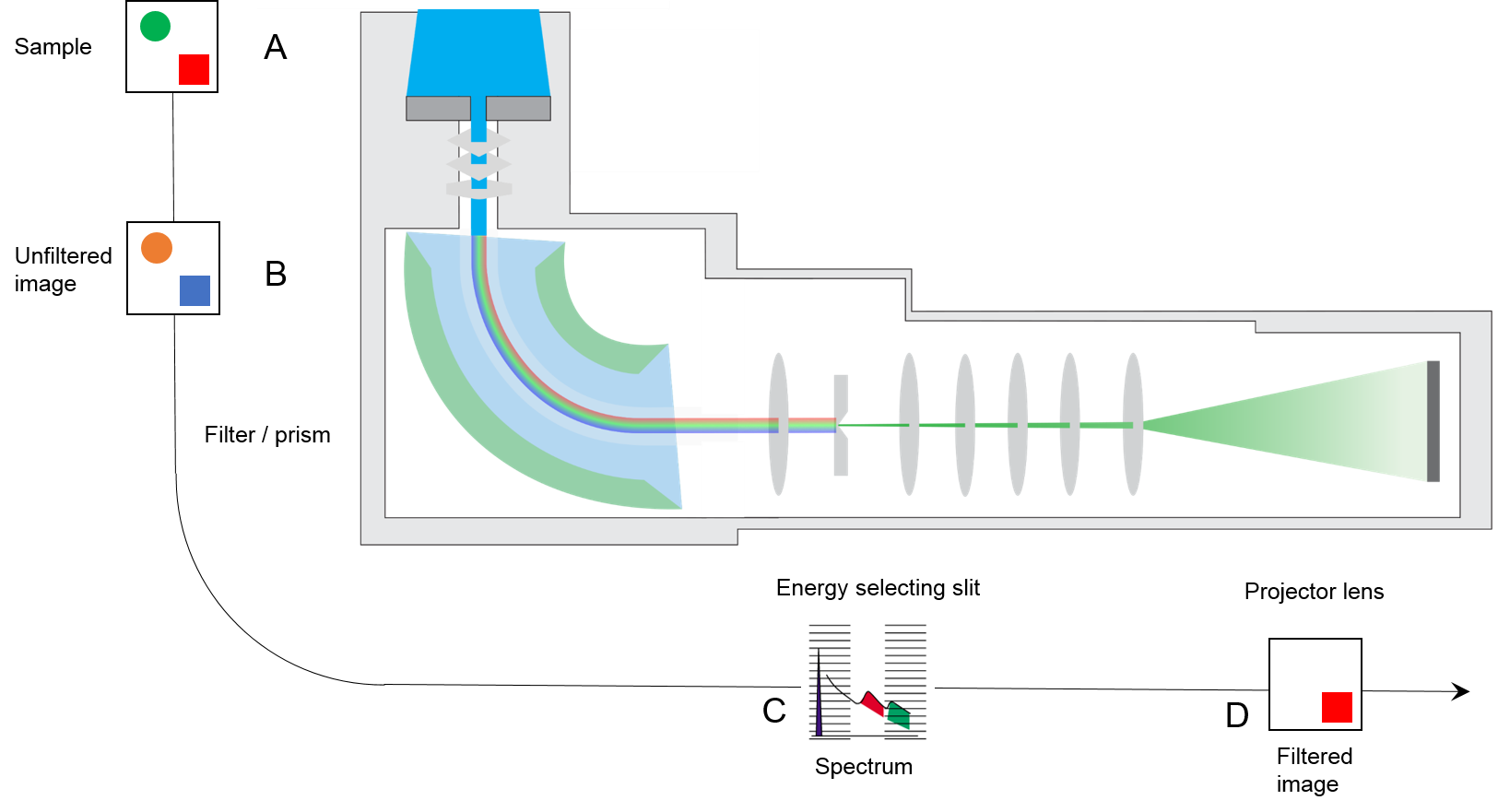
For more information on the EELS family of techniques, please visit EELS.info, an educational site.
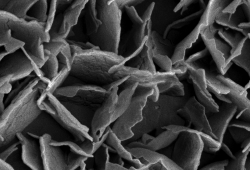
Cryo-EM
Observe biological specimens in their native environment at cryo-temperatures.
Cryo-EM - Read More
OVERVIEW
Cryo-EM is being led by the technology and innovation behind the K2 direct detection cameras.
“At present only the Gatan K2, when operated in counting mode, can produce a DQE(0) as high as 80% in conjunction with reasonably small exposure times. The K2 detector frame rate is about 10 times higher than that available with the two other detector brands.”– Subramaniam, S., Kuhlbrandt, W. & Henderson, R. (2016). IUCrJ 3, 3-7.
WHAT IS SINGLE-PARTICLE CRYO-EM?
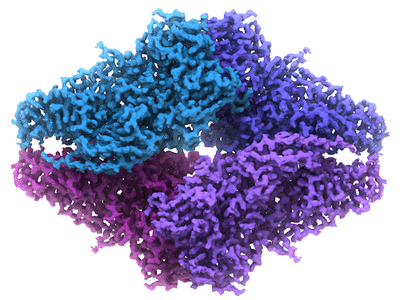
2.2 Å resolution cryo-EM structure of beta-galactosidase. Image courtesy of the Center for Cancer Research at the NIH.Single-particle cryo-electron microscopy (cryo-EM), is an increasingly popular technique used by structural biologists to solve structures at atomic resolution. This technique complements x-ray crystallography because it reveals structural details without the need for a crystalline specimen. Through the examination of a frozen-hydrated specimen in vitreous (non-crystalline) ice, the specimen ultrastructure, buffer and ligand distribution from its native state are maintained. Cryo-EM also complements structural studies using nuclear magnetic resonance (NMR) in that it enables the study of specimens larger than 150 kDa. Structural biologists frequently use cryo-EM to study viruses, small organelles, and macromolecular biological complexes, as well as molecular interactions in supramolecular assemblies or machines.
During cryo-EM, a transmission electron microscope (TEM) is used to record high-resolution images of thousands to hundreds of thousands identical, but randomly oriented, particles (molecules) from each specimen. These images are then grouped, aligned, and averaged with image classification algorithms to distinguish between multiple orientations of the 3D molecule. With a well-behaved sample, cryo-EM can solve molecular structures with a resolution below 2 Å; a resolution level that was inconceivable only a few years ago.
This rapid improvement of cryo-EM resolution has been called the “resolution revolution” and is the direct result of direct detection cameras. Conventional cameras for electron microscopy use a scintillator to convert the electron image to a light image, and a fiber optic face plate to transfer the image to a CCD or CMOS image sensor for analog recording. When the results are converted and recorded, there is a loss of high-resolution detail that has long held cryo-EM from achieving its true potential.
Direct detection cameras now directly measure the electron image to avoid the loss of detail from the conventional camera conversion steps. The Gatan K2 Summit camera is a unique direct detection camera that uses an electron counting with super-resolution technology to record the image. This technology recognizes and counts individual image electrons in real-time, while it rejects the analog read noise. As shown with the K2 Summit camera, the detective quantum efficiency (DQE) performance at half Nyquist is unparalleled at 52%; more than a six-fold improvement over conventional cameras.
EFFICIENT FRONTIER OF RESOLUTION
The K2 Summit camera, often in combination with the GIF Quantum LS imaging filter, continues to yield breakthrough results that define the efficient frontier of resolution. Cutting edge publications show these products along with improved data acquisition and image processing strategies remain at the forefront of higher resolution reconstructions for smaller molecules, such as the 2.2 Å structure of β-galactosidase.
The graph above is a comparison between the resolution and molecule size for published single particle cryo-EM structures. The K2 Summit or Quantum LS have been employed in all the structures which define the high-resolution frontier across a range of molecular sizes.
ADVANTAGES OF SINGLE-PARTICLE CRYO-EM
Now that single-particle cryo-EM provides structures with comparable resolution to x-ray crystallography, there are a number of unique advantages that are helping the technique gain traction amongst structural biologists:
Capability | Advantage |
---|---|
Examines structures in their native, hydrated state | Maintains your specimen in a biologically relevant environment, including sample and buffer concentrations |
Allows study of larger assemblies | Useful to characterize molecules larger than 150 kDa that include multiple subunits, are heterogeneous, metastable or extremely difficult to crystallize |
Elucidates atomic resolution structures | Enables observation of asymmetric side chains, hydrogen bonds and water molecules in addition to alpha helices and beta sheets |
Controls the chemical environment | Allows you to vary experimental conditions to examine molecules in different functional states |
Eliminates crystallization steps | Avoids long, uncertain preparation steps; shortens your time to publish |
Step 1: PurifyTo study molecules by single-particle cryo-EM, a specimen must be purified and structurally intact to produce high-quality 3D reconstructions. Ideally, you need to maintain the specimen in a buffer solution that keeps it biochemically active. Molecules within the specimen should be in a high enough concentration that you can study them under a microscope, but not so high that they aggregate together. Finally, experimental conditions should be optimized to promote a uniform conformational state for your molecule of interest. | |
Step 2: Plunge freezeEach specimen is frozen to prevent it from freeze-drying within the vacuum of the microscope. Nearly instantaneous freezing prevents the formation of water crystals that will disrupt the structure of the specimen.To start, you apply a small amount of the specimen in solution to a TEM grid before blotting paper is briefly used to remove excess liquid. The TEM grid is then plunged into liquid ethane to quickly wet the specimen, remove heat, and create non-crystalline or vitreous ice. The image shows the Cryoplunge™ 3 system just prior to blotting and submersion of the specimen into the liquid ethane. | |
Step 3: Transfer to TEMOnce frozen, you transfer the specimen to a specialized TEM holder that keeps it at liquid nitrogen temperature. To prevent specimen contamination, a cryo-workstation protects the specimen when you load it into the holder, then a cryo-shield encapsulates it during transfer from the workstation to the TEM. As seen here, the model 914 cryo-transfer holder is being retracted from the workstation prior to insertion into the TEM. | |
Step 4: Image specimenA specimen’s structural integrity is damaged when exposed to electrons, and typically a total dose of 10 – 30 e-/Å2 can be used before high-resolution structural information is lost. To prevent specimen damage, low dose imaging procedures are used to navigate to the desired areas and focus the TEM before acquiring images.The high DQE associated with the K2 Summit electron counting and super-resolution modes, enables you to acquire the highest quality images of delicate biological samples. This high image signal to noise ratio allows you to discern water molecules, ions and ligand structures in the 3D particle reconstruction. You can further improve image quality by utilizing the dose fractionation feature on the K2 camera, which saves full frames at up to 40 frames per second, to later correct for specimen motion and minimize drift. | |
Step 5: Analyze and reconstructOnce imaged, the Gatan Microscopy Suite® software supports your analysis and export of data into multiple formats. Data from Gatan cameras is imported into a wide variety of 3rd party software tools for 3D reconstruction and visualization, including EMAN, Frealign, Relion, and many others. The image shows a 3D single-particle reconstruction of 20S proteasome at 2.8 Å resolution. |
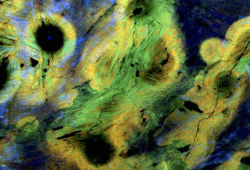
Cathodoluminescence
Unique insight into the chemical and electronic properties of materials at the microscopic level.
Cathodoluminescence - Read More
OVERVIEW
What is cathodoluminescence?
Cathodoluminescence (CL) is the emission of light when a material is stimulated by an electron beam.
Cathodoluminescence in a scanning or scanning transmission electron microscope (SEM or STEM) is a unique tool to characterize the composition and optical and electronic properties of materials, then correlate them with morphology, microstructure, composition, and chemistry at the micro- and sub-nanoscale.
Cathodoluminescence microscopy is the analysis of luminescence (emitted light or photons) from a material when stimulated by the electron beam of an electron microscope; the luminescence ranges from ultraviolet to infrared wavelength ranges.
Cathodoluminescence occurs because the impingement of a high energy electron beam elevates the sample to an excited state, which can then induce it to emit a (cathodoluminescence) photon when the sample returns to the ground state. In a semiconductor, this excitation process will result in the promotion of electrons from the valence band into the conduction band, which leaves behind a hole. Therefore when the electron and hole recombine, a photon will emit from the semiconductor.
The photon energy (color) and the probability that a photon (not a phonon) will be emitted depends on the material, its purity, and the defects it contains. Therefore to measure cathodoluminescence, you can examine almost any non-metallic material. In terms of band structure, classical semiconductors, insulators, ceramics, gemstones, minerals, and glasses can be treated the same way. In metals, the excitation of the specimen may be by surface plasmons being launched, which when they decay can result in the emission of a cathodoluminescence photon.
The electron microscope holds distinct advantages over conventional light microscopy, as the spatial resolution of light microscopy is limited by fundamental physics to an approximate resolution of 200 – 300 nm (or half the wavelength of the illuminating source). However in an electron microscope, you can focus the electron beam to a very small spot that potentially gives sub-nanometer spatial resolution. You can also use the emitted signals from the specimen to reveal morphological information, such as size and shape, as well as composition, chemistry, crystallography, electronic properties, plus much more. Thus, the volume of information from a specimen combined with the ability to correlate it directly with information from luminescence (spectroscopy) is what makes cathodoluminescence such a powerful characterization technique.
ADVANTAGES
Capability | Advantage |
---|---|
Reveals quality of semiconductor materials | Enables optimum manufacture of materials and devices; measure dislocation density |
Permits characterization of optical materials and devices | Allows investigation of optical properties at a spatial resolution better than the diffraction limit of light |
Exposes texture in minerals | Enables geochemical processes to be reconstructed by revealing trace element distributions |
Simultaneous measurement of morphology and composition | Gives a full description of a sample with direct correlation of a sample’s shape, size, crystallinity or composition with its optical properties |
Eliminates the need for complete device manufacture | Material properties can be examined in a non-destructive manner without the need for full device processing |
USES
The characterization of optical properties at extremely small length scales is critical for many areas of scientific research and technologies. Including:
|
|
Electronics and optoelectronics – In semiconductors, you can measure the local electronic band gap and reveal the defect distribution at the micro- and nano-scale. This technique allows you to easily examine direct bandgap semiconductors with strong cathodoluminescence, such as GaAs or GaN, as well as measure indirect semiconductors that emit weak cathodoluminescence, such as silicon. In particular, you can use differences in luminescence between dislocated and perfect crystalline silicon to map defects in integrated circuits. To add, the high spatial resolution provided by the focused electron beam is particularly well suited for you to examine low-dimensional semiconductor structures, such a quantum wells or quantum dots. | |
Geosciences – In rocks and minerals, observation of trace element chemistry and geochemical effects enables you to reconstruct geological processes. A SEM fitted with a cathodoluminescence detector, or an optical cathodoluminescence microscope, may be used to reveal internal structures not observable with other techniques to determine the composition, growth and provenance of minerals. | |
Material science – New sensor and communication technologies are being developed based on the interaction of light with metal nanoparticles. You can determine these properties by surface plasmons and local surface plasmon resonance modes. Recent publications show that researchers use cathodoluminescence performed in electron microscopes to study surface plasmon resonance in metallic nanoparticles at sub-diffraction limit resolutions. | |
Organic molecules – Many polymers and pharmaceutical active ingredients are shown to be cathodoluminescent. The luminescence signature is dependent on the chemical structure of the molecule rather than sample composition; hence cathodoluminescence can be used to rapidly map the distribution of organic molecules with sub-100 nm spatial resolution. |
ELECTRON MICROSCOPE SETUP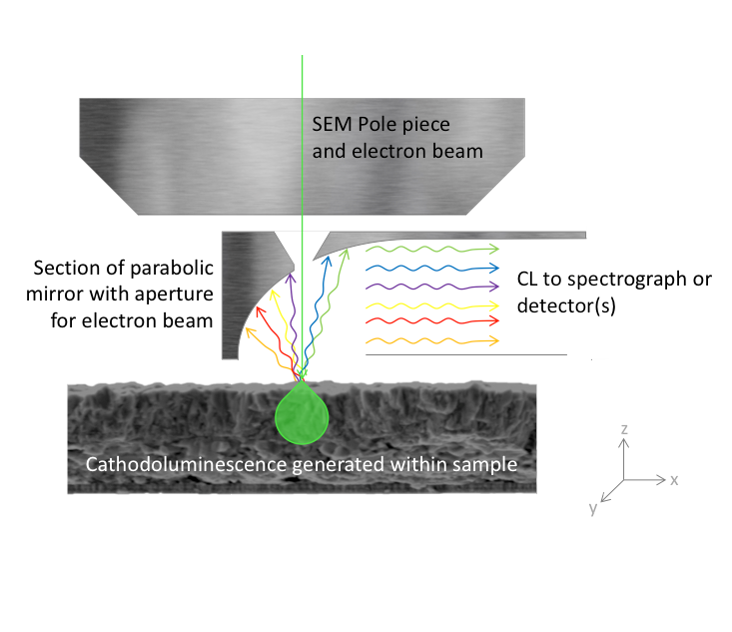
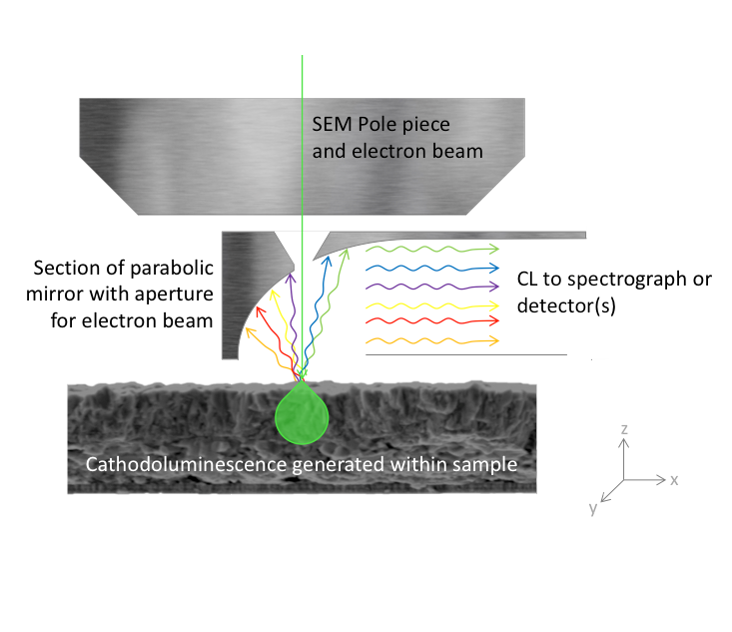
When the electron beam excites the specimen, this causes luminescence to be emitted from the near-surface region of the specimen. To collect the cathodoluminescence emitted in the upper hemisphere, a mirror is often inserted between the specimen and the pole piece. The mirror is specifically shaped to couple the light out of the microscope vacuum chamber to a spectrograph or photon detector(s). For thin samples, such as electron transparent TEM samples, mirrors above and below the specimen may be employed to collect light emitted in both hemispheres.
When you scan the microscope's focused electron beam in an X,Y pattern then measure the light emitted with the beam at each point, you can obtain an optical activity map of the specimen. The primary advantages of this electron microscope based technique is the ability to resolve features down to 1 nm and correlate the optical properties of an object with structural, compositional and chemical properties measured simultaneously or, at least within the same instrument.
Typical light levels emitted from a specimen can be extremely low and often require you to collect and detect as many photons as possible. Even in samples intended to efficiently emit light, it is important to optimize experimental conditions to collect and detect photons with the minimum of optical losses so you can attain the highest spatial resolution results.
WORKFLOW
To better understand the cathodoluminescence workflow, select the appropriate electron microscope to support you intend to use.
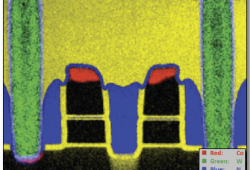
Spectrum Imaging
Systematic method to generate a spatially resolved distribution of electron energy loss spectroscopy (EELS) data.
Spectrum Imaging - Read More
OVERVIEW
Spectrum imaging (SI) is a technique that generates a spatially resolved distribution of electron energy loss spectroscopy (EELS) data. A typical experiment involves the creation of a data cube where two of the cube axes correspond to spatial information, while the third dimension represents the energy loss spectrum. The resultant dataset is referred to as a spectrum image (or spectrum line scan for the 1D case), which you can acquire and visualize in a number of ways. To create this data cube, you can acquire a complete spectrum at each spatial pixel during scanning transmission electron microscope mode (STEM SI) or collect a complete 2D image over a narrow band of energies at a single energy slice of the data cube during energy-filtered TEM mode (EFTEM SI).
A key advantage of spectrum imaging is the ability to process decisions after acquisition. When a complete spectrum is available at each data point, this allows creation of quantitative images and profiles to identify and correct data artifacts, understand image contrast, as well as determine dataset limitations.
During a STEM experiment, the electron beam focuses into a small probe, then scans over the sample to acquire spatial information (X,Y) in a serial manner. In the STEM SI mode, you can acquire a complete spectrum at each pixel position to build the spectrum image up on a spectrum-by-spectrum basis.
Alternatively, EFTEM SI uses a broad parallel beam (e.g., TEM) to acquire spectral data image plane-by-image plane, while changing the energy of each plane. In this mode, you acquire the image in parallel while the spectrum is built in a serial manner.
Once acquisition is complete, you can visualize the spectrum image (either EFTEM SI or STEM SI) either spectrum-by-spectrum (X,Y) or plane-by-plane (E). The combination of spectral and spatial information in a single dataset opens up a wide range of data analysis possibilities. You can apply any single spectrum analysis method to the entire spectrum image dataset to perform a spatially resolved spectral analysis. This increase in information provides a powerful tool for material analysis and characterization.
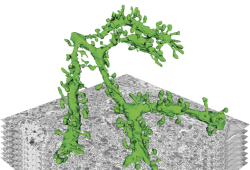
Serial Block-Face Imaging
Image three-dimensional samples from large tissue structures to thin cellular processes or organelles.
Serial Block-Face Imaging - Read More
OVERVIEW
WHAT IS SERIAL BLOCK-FACE IMAGING?
Serial block-face scanning electron microscopy (SBEM, SBSEM, and SBFSEM) is a way to reproducibly obtain high resolution 3D images from a sample. This method is particularly good at imaging large fields-of-view in X,Y,Z at nanometer resolution. SBEM typically relies on an in-situ ultramicrotome to be installed inside a scanning electron microscope (SEM). The SEM will collect an image of the block face, then the ultramicrotome will cut the sample to expose the next layer to be imaged, in steps as small as 15 nm.
ADVANTAGES OF SBEM
Capability | Advantage |
---|---|
Examines structure across orders of magnitude in scale | Maintains level of detail throughout a large dataset |
Allows high throughput with no intervention | Useful to speed up sample analysis and reduce human error |
Elucidates the context of observed ultrastructure | Enables you to gain more comprehensive results from one sample |
Controls all aspects of the sectioning and acquisition process | Allows complete flexibility to optimize for a given sample |
Eliminates section loss, damage and distortion | Avoids prohibitive processing to correct damage and distortion |
Neuroscience | Cell biology | Material science | Other |
---|---|---|---|
|
|
|
|
NEUROSCIENCE
A 3D reconstruction of a dendrite from a 15,625 μm³ (25 x 25 x 25 μm) volumetric data set containing 500 serial images of mouse cerebellum generated by the 3View® system. Dendrite structure (green), buttons (yellow), and vesicles (red). Inset images, clockwise from top left: Confocal image of a dendrite; wire frame traces rendered into a volumetric model; ultra-resolution dendritic spine model with synapses; and image showing wire frame traces.
Sample courtesy of Tom Deerinck and Dr. Mark Ellisman, National Center for Microscopy and Imaging Research, University of California, San Diego. Serial images were segmented using Imaris to create a 3D model of a neuron of interest.
CELL BIOLOGY: COMPLEMENT CONFOCAL
Image of HeLa cells, stably expressing LC-GFP grown on gridded glass bottom coverslips dishes, starved for 2 h in serum-free medium and cells of interest identified by confocal microscopy. The cells were then processed in situ for electron microscopy and the coverslips dissolved from the epoxy resin with hydrofluoric acid. The cells were again identified in the resin block and in subsequent serial images generated by the 3View system.
Samples courtesy of David Dinsdale, MRC Toxicology Unit, University of Leicester, UK.
CELL BIOLOGY: DEVELOPMENTAL
Top left: High resolution, mouse kidney, 8192 x 8192 pixel image acquired with 1.5 nm pixels. Top middle: Large field-of-view, mouse kidney, 8192 x 8192 pixel image acquired with 80 nm pixels. Right: C. elegans prepared by high pressure freezing; 4096 x 4096 pixel image acquired with 25 nm pixels. Sample courtesy of Kent McDonald, University of California, Berkeley. Bottom left: Mouse sciatic nerve, 2048 x 2048 pixel image acquired with 5 nm pixels. Bottom middle: 3D visualization of mouse sciatic nerve axons.
Samples courtesy of Gabrial Corfas, Harvard University and Children’s Hospital Boston and Kent McDonald, University of California, Berkeley. Images generated by 3View system. 3D data set contains 1,000 2048 x 2048 serial images collected with 5 nm pixels and segmented using the program Imaris.
MATERIAL SCIENCE
Upper and lower left: Images of anodized coating on aluminum surface generated by 3View system. Middle: 3D visualization of aluminum alloy with manganese particles generated by 3View system. 3D dataset contains one thousand 1024 x 1024 serial images with a pixel size of 15 nm and a cut thickness of 15 nm. 3D model created in DigitalMicrograph® using the 3D Visualization plugin.
Sample and data provided courtesy of Teruo Hashimoto and George Thompson, The University of Manchester, United Kingdom.
WORKFLOW FOR SERIAL BLOCK-FACE IMAGING
Step 1: Specimen preparationA typical biological specimen is fixed, stained with a contrasting agent and embedded in resin for stability. | |
Step 2: Mount and transfer to SEMThe specimen is trimmed to size, mounted to an aluminum pin, and optionally given a thin gold sputter coating. The specimen pin is put in the 3View system, the diamond knife is brought into contact, the door closed and evacuated. | |
Step 3: Optimize imagingThe user selects suitable beam conditions, similar to conditions for standard SEM, but also considering effects in the Z direction. Magnification, pixel count, and dwell time are chosen such that the image has the desired resolution, field-of-view, and acquisition time. | |
Step 4: Automated imagingSequential images are acquired with beam conditions of the user’s choice. Before each image, the knife removes a layer from the surface of the specimen. | |
Step 5: AnalyzeThe images are stacked to form a 3D dataset, which can be processed and viewed in DigitalMicrograph® or third-party software. At this point segmentation and quantification can be performed. |
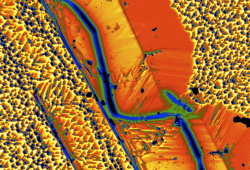
EBIC
Electron-beam induced current (EBIC) characterizes electrical properties of semiconductor materials and devices at the microscopic level.
EBIC - Read More
OVERVIEW
WHAT IS EBIC?
Electron-beam induced current (EBIC) technique enables the characterization of local electrical behavior of semiconductor materials and devices by measuring the electrical currents that flow when a sample or device is exposed to an electron beam.
When an electron beam strikes a semiconductor, electron-hole pairs are created. If the carriers, of said electron-hole pairs, diffuse into a region with a built-in electric field, the electrons and holes will separate and a current will flow. The EBIC technique measures that current when it flows into an external circuit. In a material with no recombination centers (locations where the free electrons and holes annihilate) the collected current would be uniform and of little interest. However, regions of the sample which cause electrons and holes to recombine, reduce the collected current to cause contrast in an EBIC map, thus reveal the flow of (minority) carriers in a semiconductor sample.
WHAT IS EBAC?
Electron-beam absorbed current (EBAC) is a related technique where the local resistance of a sample is revealed when you measure the absorbed current that flows between the electron beam and an electrical probe. The current measured in the external circuit is proportional to the resistance of the electrical path travelled. Thus, EBAC reveals breaks in copper lines on electronic devices or, shorts and breakdown channels in capacitors.
ADVANTAGES OF EBIC AND EBAC
Capability | Advantage |
---|---|
Examines p-n junction and depletion region locations | Measure the effectiveness and uniformity of device fabrication |
Elucidates the impact of recombination centers such as grain boundaries, dislocations, and precipitates | Enables optimization of processing steps to maximize device efficiencies |
Measures minority carrier diffusion lengths | Allows you assess material quality with high spatial resolution |
Reveal line breaks in integrated circuits | Minimizes the time to diagnose fault locations |
Step 1: Prepare the sampleThe standard EBIC technique requires the specimen to contain an electrical junction with diode characteristics – this provides the electric field to separate electrons and holes – and ohmic contacts, to allow connection to an external circuit. Fortunately, many devices such as solar cells, laser diodes, and transistors are suitable for analysis without further preparation; the user simply needs to ensure that the region of interest is accessible to the electron beam. For examination of material prior to device fabrication, you can form the diode junction by depositing a thin, electron transparent, metallic Schottky barrier character on the region of interest and a second ohmic junction to allow connection to the external circuit. | |
Step 2: Transfer to scanning electron microscope (SEM) and verify electrical contactIn order to measure the small currents associated with EBIC or EBAC experiments, electrical contact must be made to the specimen. This is done frequently via a dedicated EBIC holder by wire bonding or the use of adjustable micromanipulators. A current-voltage trace is frequently acquired to analyze the diode-characteristics and determine if the specimen is suitable for EBIC. For quantitative EBIC measurements, the leakage current in reverse bias and shunt resistance should be low.An EBIC holder and sample may be loaded onto the SEM stage through the load lock of many modern SEMs; the stage will have been modified to contain mating electrical connections that allow the EBIC or EBAC signal to be passed to the external measuring circuit. Verification of good electrical contact can again be made by acquiring an IV trace. | |
Step 3: Image specimenAs the electron beam is scanned across the specimen in an X,Y pattern, you can measure the collected current at each pixel. In the simplest setup, you can use an arbitrary grayscale to represent the magnitude of the current; conventionally small and larger currents are displayed in black and white, respectively. Although qualitative imaging is useful to reveal the location of electrical junctions, you can perform a more meaningful analysis through quantitative measurements. In a quantitative measurement, the grayscale levels in the image display are associated with measured currents (typically pico- to micro-A range), rather than arbitrary units. This enables meaningful comparison between samples and a true understanding of the impact of (for example) defects. | |
Step 4: AnalyzeYou may analyze the electrical properties in absolute intensity current values. However the EBIC contrast is a more useful parameter to measure impact of defects, such as dislocations, grain boundaries, and stacking faults. This measures the current normalized by the background signal, and relates directly to the recombination strength of a defect. It is also possible to measure minority carrier diffusion lengths locally through analyzing the EBIC profile caused by the diffusion of minority carriers to an electrical junction. |
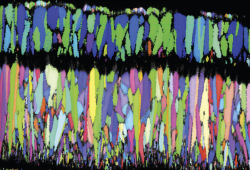
EBSD
Electron backscatter diffraction (EBSD) helps you examine crystallographic orientation or texture of materials.
EBSD - Read More
OVERVIEW
WHAT IS EBSD?
Electron backscatter diffraction (EBSD) is emerging as a valuable analytical tool for materials science and earth sciences. The EBSD technique permits statistical data on grain size and grain texture, both of which are extremely important parameters in determining the strength of crystalline materials. A good general reference on all aspects of EBSD can be found at www.ebsd.com
The reflected signal from the sample surface used in EBSD is generated within the first 10’s nm of the sample surface. Thus it is critical to have a surface that is near perfect, with little or no damage. Broad argon beam tools have historically been used to produce damage-free transmission electron microscope (TEM) samples. The same techniques are now applied for scanning electron microscope (SEM) samples used to produce EBSD signals.
IPFZ map of stressed Zircaloy 2 alloy, FOV 1 mm x 0.7 mm. Results courtesy of Department of Materials, University of Oxford. Professor Angus Wilkinson and Dr. Hamidreza Abdolvand. Data acquired on a Zeiss Compact Merlin equipped with a Bruker Quantax EBSD System.
USES
Crystal orientation | Grain size |
Local & global texture | Recrystallize/deformed fractions |
Substructure and strain analysis | Grain boundary characterization |
Phase identification, distribution, and transformations | Fracture analysis |
3D EBSD WITH BROAD ARGON BEAM TOOLS
This can be done manually by returning the sample to the PECS II instrument and polish removing from 5 – 500 nm simply by varying the time. An example is shown below.
WCarbide sample 140 x 140 x 15 µm3, 25 slices 600 nm thick. Image and data courtesy of University of Manchester.
A recent development by Gatan is to automate this process by integrating a PECS II instrument onto a FIB/SEM. The system was installed in November, 2015 and data will be published shortly.
WORKFLOW
Step 1: Prepare the sample
Schematic outline of iPrep II system attached to a SEM.
Specimens must be prepared carefully for EBSD applications, as the technique requires a very smooth specimen to avoid shadows on the diffraction pattern. The best way to accomplish this is to utilize a fully automated argon ion polishing system, like the PECS II instrument. By using a PECS II instrument, you can produce damage free surfaces, cross sections, and deposit coatings to protect or eliminate charging. Typical conditions for planar polish in the PECS II are the guns set to 2 – 4° to the surface with a coarse polish at 4 – 6 keV for 1 – 2 h, followed by a lower polish voltage of 1 keV for 30 – 60 min.
Step 2: Transfer to SEM
Schematic of iPrep II system shows transfer of sample to home position on a SEM stage.
Step 3: Imaging
Schematic of iPrep II system shows the sample on a SEM stage with the transfer rod retracted. SEM and sample now in position for SEM analysis.
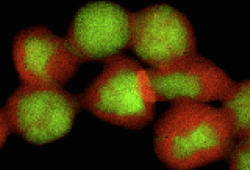
EDS/EDX
Energy dispersive x-ray spectroscopy (EDS/EDX) helps you to elucidate elemental or chemical characterization of a sample.
EDS/EDX - Read More
OVERVIEW
WHAT IS EDS?
Energy dispersive x-ray spectroscopy (EDS, EDX, XEDS, etc.) is an analytical technique used for analysis and characterization of a sample.
Elemental composition analysis is key to understanding foreign materials, coating composition, small component materials, rapid alloy identify, evaluating corrosion, plus phase identification and distribution. EDS tools from Gatan provide qualitative and quantitative insight, in addition to elemental maps for microstructure determination during these types of material studies.
Since the changing local chemical environment atomic bonding has little effect on EDS, it is not a preferred technique for specimen chemical analysis. However, it is excellent to determine the elemental distribution of a sample. Used in combination with electron energy loss spectroscopy (EELS), you can easily analyze both the chemical and compositional makeup of your specimen.
Unlike similar techniques, EDS requires minimal setup and is used in semi-thin to bulk specimens; thickness options are unlimited. EDS also offers a high signal-to-background ratio (SBR). Some limitations of EDS include: non-local fluorescence; low-Z limits; and limited thin film signal-to-noise ratio (SNR).
In the example below, the Sr L2,3-edges at 1940 eV were not within the 0 – 850 eV energy range (top) after an EELS analysis was run on a semiconductor sample. When spectrum imaging was used to obtain the EDS Sr elemental map in combination with the EELS Ti, Fe, and La maps (bottom), the collective color map shows the distribution of each element across an extended energy range.
Total exposure time: 118 s; EELS core-loss: 350 – 850 eV; EELS low-loss: 0 – 500 eV; EDS spectrum: 0 – 20 keV beam current: 200 pA.
Sr EDS: red; Ti EELS: green; La EELS: orange; Fe EELS: light blue.
For more information about the comparison between this technique and other analytical techniques, please visit EELS.info, an educational site.
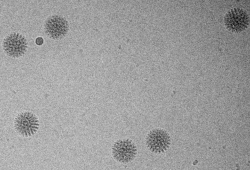
Specimen Holders
Cool, heat, strain, tilt, or transfer (cryo- and vacuum) samples to help you understand behavior under environmental conditions.
Specimen Holders - Read More
OVERVIEW
What is in-situ microscopy?
In-situ transmission electron microscopy combines the image formation capabilities of the transmission electron microscope (TEM) with application of some external stimulus to measure results real-time. Currently, a wide variety of systems and holders are available to apply different stimuli to evaluate kinetic reactions such as catalysis, phase transformations, other chemical reactions, electrical fields, mechanical strain or nano-indentation deformation, heating and cooling, or the effects of the electron beam irradiation damage in samples.
Historically, movies of various reactions and system kinetics could be recorded onto video tape. More recently screen capture programs allow low-resolution, qualitative capture for you to visualize what is happening at video frame rates, e.g., 30 frames per second (fps). This adds significant value to investigators who want to physically see and understand reactions in real-time.
With the advent of faster data transfer and processing capabilities, it is now possible to record and manage large datasets directly from the sensor output. You can store each pixel in each frame directly to disk, and treat them as an individual image. Alternatively you can apply various algorithms or scripts (e.g., summing, drift correction, binning, etc.) post-capture to quantify the stimulus applied to the system. This greatly enhances the flexibility of data management and gives you previously unseen resolution in time and space, with sub-ms time resolution to resolve reactions that previously were unknown.